The Apple Of My Eye: A New Model For Eye Regeneration Studies (Journal Club #1)
In this inaugural "journal club," we discuss a recent pre-print that aims to establish the golden apple snail as a model for eye regeneration studies.
Introduction
Could a snail hold the secrets to eye regeneration? Researchers at Stowers Institute are aiming to find out. Led by Stowers postdoc (and soon-to-be associate professor at UC Davis) Alice Accorsi, the scientist and her colleagues are developing the golden apple snail (Pomacea canaliculata) as a model for eye regeneration studies. Before diving into the science, however, let’s first review the significance of this model and what’s been accomplished in eye regeneration thus far.
Traditionally, biology has relied on a few select models for the study of many different themes. These models include E. coli, baker’s yeast (Saccharromyces cerevisae), fruit flies (Drosophila melanogaster), mice (Mus musculus), nematodes (Caenorhabditis elegans), chick (Gallus gallus), and the zebrafish (Danio rerio). These organisms have become widely used for the several advantages they confer, such as genetic tractability (in other words, the ability to manipulate the genome successfully), economic feasibility (the means to maintain and care for the organisms), and unique characteristics specific to the organism (for example, the zebrafish embryo is optically clear, making it especially amenable to live imaging and visualization).
While these organisms have contributed much to many fields of biology, the past decade or so has seen a surge in the inclusion of new non-traditional model organisms. In relying on a small number of organisms from select parts of the phylogenetic tree, biologists felt that we were limiting our understanding of biological trends and processes as well as the evolutionary contexts underlying them.
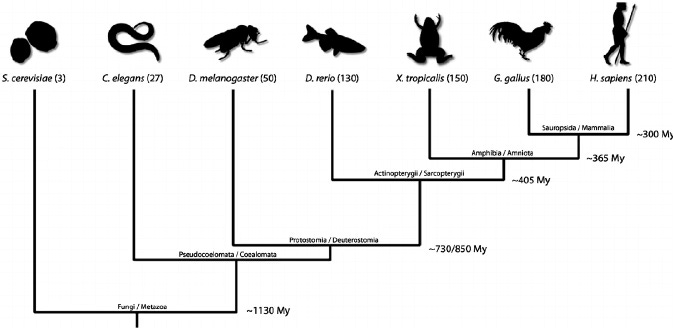
Now, biologists are attempting to develop models from all areas of the biological tree to both better address phylogenetic gaps and to ask questions that cannot be investigated with existing models. In this case, the golden apple snail represents a perfect union of both these goals.
Vertebrates, including humans, have what is termed a camera-type eye. Light first enters our eye through the cornea, which focuses and refracts it into our pupil. Here, the iris acts as a shutter of sorts, controlling how much light enters the pupil. Light then enters the lens, which focuses the light, and continues onto the retina. The retina takes the light input and converts it into information that can be sent to the brain via the optic nerve.
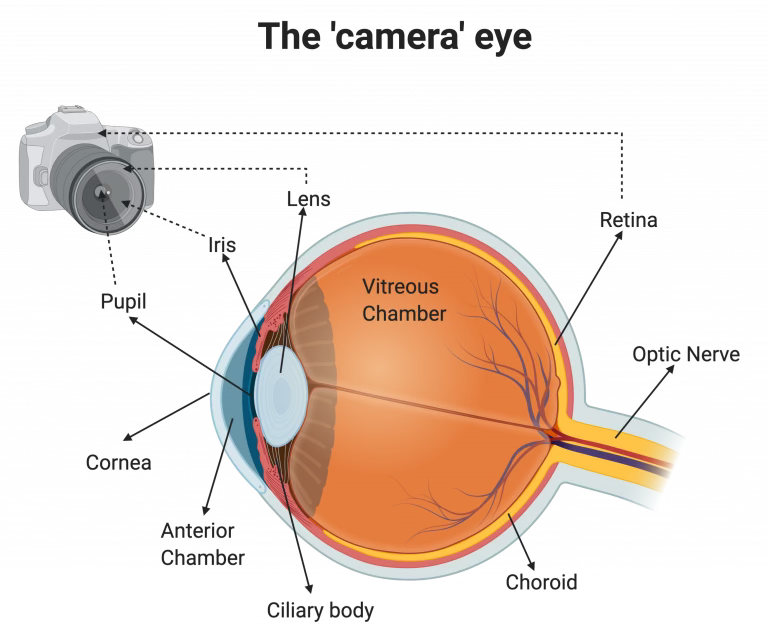
The camera-type eye is a complex system with multiple components, which can be difficult to model completely through an organism. While there have been multiple vertebrate models developed for the study of camera-type eye regeneration, such as the zebrafish, frog, and newt, these organisms can only regenerate certain structures within the eye, such as the lens and retina. Additionally, while other models with different eye types–such as planarians, or flatworms–have been used to attempt to model complete eye regeneration, their simpler eye types make it difficult to apply results to a human or vertebrate model.
This is where the golden apple snail takes the stage. Other groups of animals, such as crustaceans and molluscs, also have camera-type eyes. However, not all of these animals have regenerative abilities. Snails, then, are unique in that they possess camera-type eyes that can be completely regenerated, positioning them as a singular model for the study of camera-type eye regeneration. In this preprint, the authors detail their journey towards establishing P. canaliculata as a tractable model in the lab and their characterization of camera-type eye regeneration in the snail.
Methods Breakdown
This publication makes use of the following experimental techniques: histology (H&E staining), scanning electron microscopy (SEM), transmission electron microscopy (TEM), cell proliferation assays (more specifically, H3P antibody staining and BrdU pulse chase experiments), and transcriptomics. For those who are unfamiliar with these techniques, either on a broad conceptual level or on a more technical level, this section will give a brief overview of what these techniques are used for and how they work.
Histology
Histology is a technique that allows scientists to see the microstructures of a particular piece of tissue. It involves sectioning the tissue, staining it, and examination of the different structures and characteristics of the tissue. You may be more familiar with histology in the context of pathology (i.e. diagnosing diseases), but it’s widely used in biological studies, especially ones that aim to characterize tissues that have not been described previously. One of the most common histological stains is known as H&E staining. This technique uses hematoxylin, which stains cell nuclei, and eosin, which stains the cytoplasm and extracellular matrix. These stains allow researchers to visually distinguish the different layers and structures of their tissue of interest, which can provide them with valuable information–in the case of this paper, the authors’ use of H&E staining allows them to draw comparisons between the eye of their new model and the vertebrate eye, for example.
Scanning Electron Microscopy (SEM) & Transmission Electron Microscopy (TEM)
While multiple types of microscopy are based on light, SEM & TEM take a different approach to visualization of a biological sample. These microscopes use an electron gun to “shoot” electrons (the negatively charged components of an atom) at the sample within a vacuum. When the electrons make contact with the sample, their interactions with the different atoms composing the sample generate secondary electrons, back-scattered electrons, and x-rays. In the case of SEM, different detectors collect these secondary products and produce an image of the surface of the sample. TEM, however, actually transmits its electrons through the sample (which must be ultra-thin), allowing for visualization of the sample’s internal structures. Both forms of microscopy provide extreme magnification up to a couple million times, which can be incredibly useful for the visualization of microscopic structures.
Cell Proliferation Assays (H3P and BrdU pulse-chase)
Previous work has identified phospho-histone H3, a structural protein found in DNA, to have important roles in cell-cycle progression and mitosis. Because of this, scientists use it as a marker for visualization of cell proliferation (i.e. increased cell divisions) in multiple contexts, from cancer to regeneration. In this paper, the authors used an antibody that targets H3P to be able to visualize cell proliferation throughout regeneration. They used this antibody in combination with a bromodeoxyuridine (BrdU) stain also used as a measure for cell proliferation. On a chemical level, BrdU resembles the nucleoside thymidine (not to be confused with the nucleotide thymine, or T), which is thymine paired with a deoxyribose sugar (what makes up the backbone of DNA). This means that when DNA is being synthesized (a step that precedes mitosis), BrdU is incorporated into the new DNA, which can then be targeted using an antibody. This stain is typically applied in a “pulse-chase fashion” where the BrdU is introduced (pulse), followed by an incubation period (chase) before fixation of the sample. After binding of these primary antibodies, a secondary antibody that has a fluorescent molecule attached to it will bind to the primary antibodies, allowing us to visualize these different markers using tools like confocal microscopy.
Transcriptomics
Transcriptomics refers to the study of gene expression in a given sample. These datasets are generated using different methods of next-generation sequencing. In this work, the authors use RNA-seq, a method of sequencing that captures and quantifies transcripts from regenerating eye tissue. Briefly, RNA is extracted from a sample, prepared for sequencing using multiple techniques (fragmentation, development of a cDNA library, etc.), and sequenced in specialized machines that can generate a dataset with all of the reads (i.e. nucleotide sequences) found in the sample. This is a difficult concept for even seasoned biologists to fully comprehend, so feel free to reference the figure below for clarity. However, all you really need to understand for this paper is that RNA-sequencing allows us to see gene expression and dynamics in key populations of tissue and at varying time points.
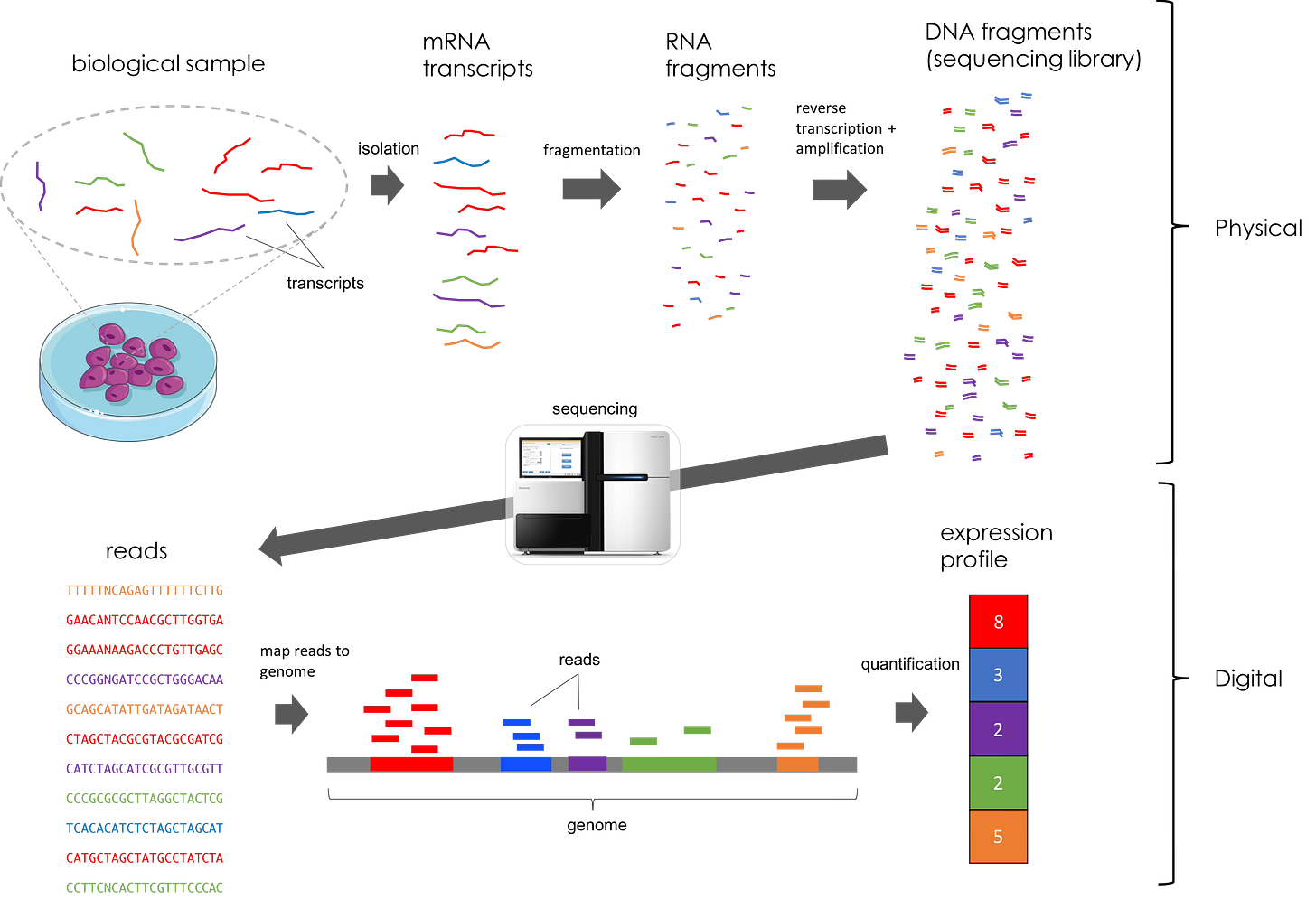
The Science
Introduction
You may be wondering, how did researchers know to target an organism like the golden apple snail for these kinds of studies? Previous scientific and descriptive work (some of it even dating back to the late 1700s!) had already indicated the potential for gastropods, or snails and slugs, as a model for eye regeneration [1-3]. While the golden apple snail is primarily known for its invasive nature as a rice pest, the lab that produced this work, the Sanchez-Alvarado lab at Stowers Institute, began developing resources for the snail as part of its efforts to develop new research organisms for biology [4]. They sequenced the genome of P. canaliculata–an important first step towards genetic tractability–and established the husbandry necessary to successfully keep the snails in the lab [5]. Other advantageous traits, such as the diploid nature of the organism (in other words, having two sets of chromosomes like us), further cemented the snail’s promise. After having completed the groundwork, the authors set out to assess P. canaliculata’s potential as a model for camera-type eye regeneration.
Below, you’ll find a question-by-question breakdown of the authors’ approach, the techniques they used, and what they found. Feel free to pull up the pre-print and follow along!
Question: Is the anatomy and structure of the P. canaliculata eye comparable to that of the vertebrate camera-type eye?
Method(s): dissection, histology, TEM
In order to ensure that their model would be able to provide important insights into camera-type eye regeneration, the authors first needed to see whether the P. canaliculata eye had a comparable anatomy and structure to that of the vertebrate camera-type eye. Additionally, they needed to be sure that experimental manipulation of the eye was feasible before moving on towards more complex assays. Through dissection and examination, the authors were able to isolate the retina, optic nerve, and lens of the P. canaliculata eye. They also showed that the snail lens is able to invert and focus an image, just as vertebrate lenses do. To get at the anatomical level of the eye, the authors used H&E staining to visualize the different tissues and tissue layers that compose the P. canaliculata eye. They observed the presence of a cornea, an anterior and posterior chamber, lens, the different retinal layers, and the axons that compose the optic nerve. From there, they used TEM to visualize the different cell types making up the eye, particularly within the lens and retina. While the authors did observe some characteristics more indicative of invertebrate eye anatomy, they ultimately concluded that P. canaliculata does have a complex camera-type eye structure that shares some anatomical characteristics with vertebrate camera-type eyes.
Question: Can P. canaliculata successfully regenerate its eye? What does the overall timeline of regeneration look like?
Method(s): histology, cell proliferation assays (H3P, BrdU pulse-chase)
Having examined the comparability of the P. canaliculata eye to the vertebrate camera-type eye, the authors next wanted to assess the regenerative ability of these eyes. They began by amputating the eye stalk and observing the snails, noting that complete regeneration of the eye took about a month. To understand the cell proliferation underlying this process, as well as the morphological changes occurring during regeneration, they used histology and cell proliferation assays in tandem. Using these techniques, the authors defined four key stages of eye regeneration: wound healing (which occurs within the first 24 hours), blastema formation (between 3-6 days post amputation, or dpa), retina and lens emergence (between 9-12 dpa), and eye component maturation (between 15-28 dpa). Interestingly, they found that the different eye components–such as the lens, different photoreceptors, and optic nerve axons–appear at different points during eye regeneration and grow at different rates. Additionally, the regenerated eye at 28 dpa is smaller than it was pre-regeneration, suggesting that additional maturation and growth may occur following the completion of regeneration.
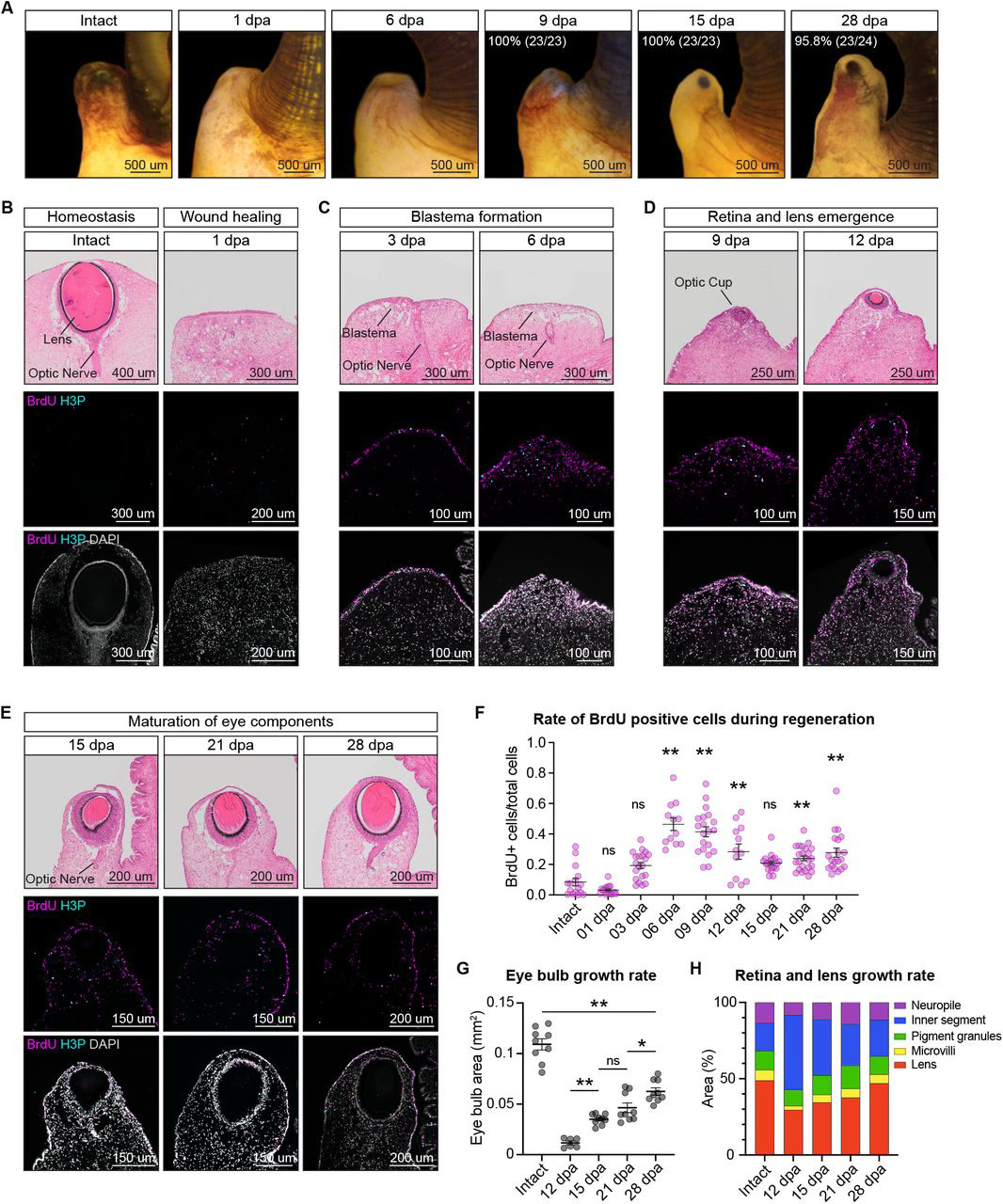
Question: How does gene expression change throughout eye regeneration?
Method(s): sequencing, transcriptomics
Having completed a morphological and cellular analysis of the regenerative timeline, the authors wanted to understand how gene expression changed during eye regeneration. They took samples of an intact eye and of the regenerating eye at their previously defined timepoints (1 dpa, 3 dpa, 6 dpa, 9 dpa, 12 dpa, 15 dpa, 21 dpa, and 28 dpa) and sequenced them, subsequently generating sets of RNA transcripts for each sample. Using a form of computational analysis called gene ontology (GO) term analysis, the authors assessed what biological processes and functions these transcripts were associated with.
Within the first 24 hours following amputation, genes involved in the cell-cycle were enriched (in other words, had higher expression levels compared to other time points), a result that was in agreement with their observations on cell proliferation following injury. By the blastema stage (3-6 dpa), genes associated with camera-type eye development were being expressed alongside genes associated with neuron production, almost like a “reboot” of the eye’s genetic identity program. Towards the emergence of the different eye components, genes associated with photoreceptors and visible light are enriched, eventually giving way to genes associated with visual perception. For the authors, this result seemed to agree with the variation they observed in terms of the growth and maturation of these different elements.
Question: Can we build the tools necessary to be able to experimentally manipulate the P. canaliculata genome?
Method(s): ex ovo culture, microinjection
Having laid down the groundwork for characterization of eye regeneration, the authors wanted to develop tools that would enable them to perturb and further probe eye regeneration in P. canaliculata. To collect and culture the embryos, they had two main obstacles they needed to overcome: internal fertilization and the necessity of perivitelline fluid (an extraembryonic fluid that partially isolates the embryo from the environment) for development. To work around these constraints, the authors would collect clutches as soon as they were laid by the snails and open them up, subsequently transferring them to a petri dish with a perivitelline fluid extract they developed. This way, the embryos would continue to develop outside of the clutch, meaning that they can be observed and manipulated independently.
Typically, genome manipulations like knock-outs are done at the one-cell stage in embryos. This is because, as cells divide, they will maintain the manipulation in their genome, meaning that all the cells in the organism will have the given manipulation. However, because embryos are incredibly small (the P. canaliculata embryo, for example, has a diameter of 100 microns), scientists must use special tools to be able to deliver material to the embryo. In most cases, scientists will use a micro-injection rig, which inserts a glass needle into the embryo and injects the embryo with the molecular ingredients necessary to induce the genetic manipulation. While the authors had to adjust their set-up over multiple rounds of micro-injection, they were able to successfully inject exogenous (as in, not belonging to the organism) mRNA encoding fluorescent proteins, which they confirmed by observing fluorescence in the embryo itself.
Question: What does pax6 expression look like during development and regeneration? What happens when it is functionally perturbed?
Methods: transcriptomics, HCR, CRISPR-based mutagenesis
After successfully establishing the tools necessary for genetic manipulation of P. canaliculata, the authors wanted to put these tools to the test and assess the expression and function of a conserved eye development gene: pax6. While the snail has five pax6-like genes, only one showed significant conservation and the presence of a complete PAX domain (i.e. the most functionally important component of this gene): LOC112559942. Additional phylogenetic analyses found that this gene was most similar to both human and fly pax6 genes, indicating that it may be a potential ortholog, or a gene that has evolved from a common ancestral gene and retains a similar function.
The authors once again used a sequencing and transcriptomics approach to understand pax6 expression both during development and regeneration. They found that expression of pax6 increases within the first 6 days of regeneration and that pax6 is generally more highly expressed in the adult retina. To assess expression of pax6 during development, the authors used a technique called in situ hybridization chain reaction (HCR). This technique allows for the visualization of mRNA by hybridizing a complement (also referred to as an anti-sense probe) to the transcript of interest. Fluorescent signal is generated when molecules with fluorophores (“hairpins”) attach to the probe and amplify. They found that pax6 expression could first be seen at 3 days post-fertilization (dpf) in the anterior part of the embryo. Over the next few days of development, expression was observed in the eye buds, gradually becoming confined to the eye stalk.
In addition to characterizing the expression of pax6, the authors also wanted to test its function. To do this, they used CRISPR-Cas9 to generate a mutant snail lacking pax6 expression (also known as a loss of function mutant). They found that, at 9 dpf, the snails did not have a retina or eye stalk. In fact, they couldn’t find anything resembling an eye structure. Additional staining with a phalloidin antibody that labels actin, a protein that forms structural components in the cell, further showed that there was no eye formation occurring in the absence of pax6. The authors also observed a change in behavior from these mutant snails; unlike their wild-type (i.e. normal, or non-mutant) counterparts, these snails were unable to rotate, instead lying on their side at the bottom of the tank. However, wild-type snails whose eyes were amputated did not engage in this behavior, which led the authors to assume that pax6 also encodes a component relevant for locomotion or gravity perception.
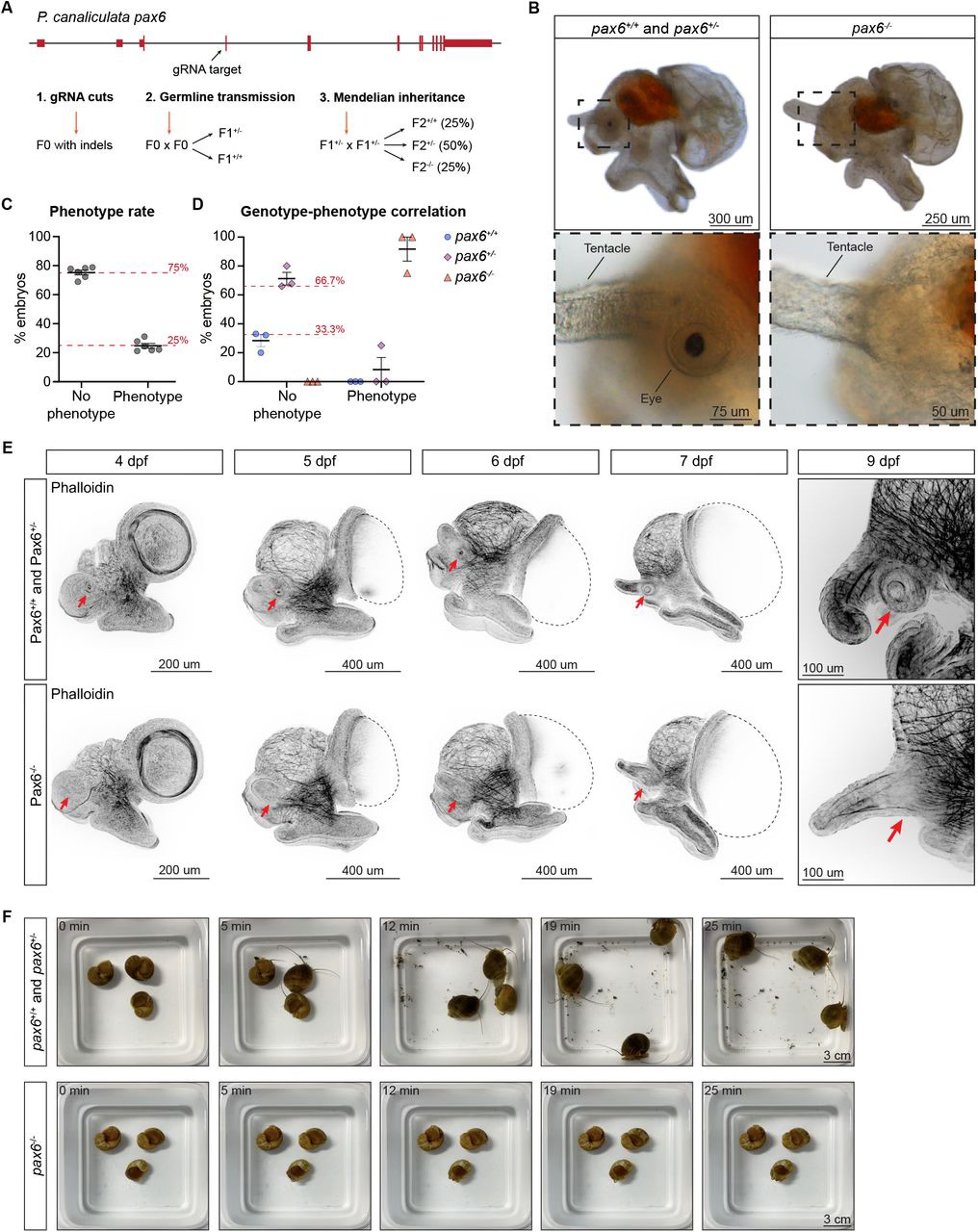
Discussion
As a developmental biologist with interests in unconventional models and evo-devo (or, the evolutionary side of developmental biology), I find efforts to establish new models incredibly important and impressive. While much has been accomplished in biology using traditional model organisms, we will need to take advantage of the full range of biodiversity if we hope to both uncover how widespread different biological trends are and to characterize unique biological processes that exist beyond that of our traditional models.
To me, this manuscript presents an incredibly comprehensive effort towards the establishment and development of the golden apple snail as a model for eye regeneration studies. The authors develop a slew of tools–a genome, culturing protocols, microinjection, and CRISPR-based mutagenesis–that will allow researchers to design and perform a variety of experiments from the get-go. Additionally, as the model becomes more established, more tools may come along that will continue to expand the kinds of questions we can ask and test experimentally. Given that the golden apple snail is the only current model that can model complete camera-type eye regeneration, this work also marks an important step towards characterizing that process and identifying potential therapeutic targets down the line.
In terms of the science, I believe the authors took an unbiased and detailed approach to their characterization of the snail and its regenerative process. They did not jump to any conclusions and acknowledged that further work would be needed before reaching a firm interpretation of their initial results. I will be curious to see how P. canaliculata’s regeneration process could be applied to human eye research given that it has characteristics of both invertebrate and vertebrate eyes, but this will take further study to answer. Additionally, I also wonder what the feasibility of other common tools, such as transgenesis, will be for this particular model. Overall, however, the model shows a lot of promise, and I am eager to see what answers we will be able to derive using it.
This effort is all the more impressive due to the risks it took. While funding is a difficult endeavor no matter the project, funding agencies are usually hesitant to fund projects they believe to be “high-risk.” Getting a model up and running, especially one that has not had genetic tools developed yet, is an effort that agencies may not consider feasible, making it more difficult to fund projects such as these. The commitment and thoroughness that these researchers show is commendable, and I hope that their success inspires both other researchers and funding agencies to continue prioritizing the development of non-traditional models, particularly those that could answer questions we cannot ask with our current models.
Overall, this work sets the stage for the use and further development of a model that fulfills a research niche with a lot of future potential, especially as it pertains to human eye regeneration. I can’t wait to see how this research continues to evolve in the future, and I hope more work like it is on the way!
References
[1] Spallanzani, L., Dalyell, J. G. & Bonnet, C. Tracts on the Natural History of Animals and Vegetables. vol. v.1,2d ed (Printed for W. Creech and A. Constable; Edinburgh, 1803). https://doi.org/10.5962/bhl.title.10539
[2] Bever, M. M., & Borgens, R. B. Eye regeneration in the mystery snail. J. Exp. Zool., 245:33–42 (1988). doi:10.1002/jez.1402450106.
[3] Tuchina, O., & Meyer-Rochow, V. B. Regeneration of the visual system in gastropods (Mollusca). Invertebrate Biology, 129:27–38 (2010). doi:10.1111/j.1744-7410.2010.00195.x.
[4] Sánchez Alvarado, A. To solve old problems, study new research organisms. Dev Biol, 433:111–114 (2018). doi:10.1016/j.ydbio.2017.09.018.
[5] Sun, J., Mu, H., Ip, J.C.H., Li, R., Xu, T., Accorsi, A., Sánchez Alvarado, A., Ross, E., Lan, Y., Sun, Y., et al. Signatures of Divergence, Invasiveness, and Terrestrialization Revealed by Four Apple Snail Genomes. Molecular Biology and Evolution, 36:1507–1520 (2019). doi:10.1093/molbev/msz084.